Energy is considered the cornerstone of economic growth [1]. The continued industrialization and growth of the global economy are dependent upon the availability of energy sources to power our planet. However, every energy conversion and transmission activity affects the environment. The use of fossil fuels can pollute land, air and water and can impact wildlife and the landscape. Renewable energies are the alternative solution to the social and environmental challenges caused by fossil fuels and nuclear energy, it provides a sustainable solution and advantage over traditional energy sources and contributes to the Sustainable Development Goals (SDG), adopted by the United Nations in 2015 [2]. The seventh (SDG) ensures access to affordable, reliable, sustainable, and modern energy for all-includes a target to increase the contribution of renewable energy to the global energy mix [2]. To cope with energy demand growth, pollution, energy security, and climate change [3] [4] [5] [6] governments through the globe, set ambitious targets for the utilization of renewable energies and reduction of gaseous and particulate pollutants. These targets continue to be important drivers of the expansion of solar and wind power deployment worldwide.
This paper provides an overview of the onshore and offshore wind energy and solar PV and CSP energy development. Also, it is comprehensively synthesizing the current knowledge about the environmental impacts of these two important renewable energy sources. This review analysis is of value to cover and analyze, as these environmental impacts are commonly used and should work in harmony with the planning and commissioning stages of solar and wind energy projects. Hence, analyzing the environmental impact provides guidance, insight and significant opportunity to researchers, developers, decision-makers, and communities to diffuse and adopt solar and wind energy projects. Our review provides a tool that helps to better understand the relevant factors that drive and impede the wide adoption of the renewable energy sector and therefore
In general, there are two major categories of solar energy technologies; photovoltaics (PV) and concentrating solar power (CSP). PV cells convert solar radiation directly into electricity, whereas mirrors are used in CSP to reflect and focus sunlight to generate heat and convert it into power. Solar energy installations can be distributed or large scale depends on the size and location. Distributed solar energy systems (typically ˂1 MW) are independent of the grid and they are often building integrated. Large scale solar installations are geographically centralized and sometimes built far away from where the power is needed, e.g. in deserts where the solar irradiation is high and the population density is low [7].
Solar energy is a great alternative to carbon-intensive energy sources and has enormous potential to minimize polluting emissions and thus contribute to climate change mitigation [8]. Zhai et al. [9] found that the total amount of avoided CO2 emissions can range from 6.5% to 18.8% if 10% of the power in 10 different states in the US were generated from PV.
Solar PV power generation plays a significant and growing role in many countries. The annual global market for solar PV increased by 100 GW in 2018 to reach the total installed capacity of 505 GW [10]. Figure 1 shows that the majority of global solar development is in the PV sector. In 2018, the CSP counted only for less than 1.1% (5.5 GW) of the total global installed capacity. By the end of 2018, 640 TWh of PV electricity was generated worldwide, which is 2.4% of the annual global electricity generation [10]. In developing countries, off-grid solar PV systems continue to contribute to the electrification of rural areas. It is believed that 5% of the people in Africa are powered by off-grid PV systems [10].
The global installed PV and CSP capacity (GW)
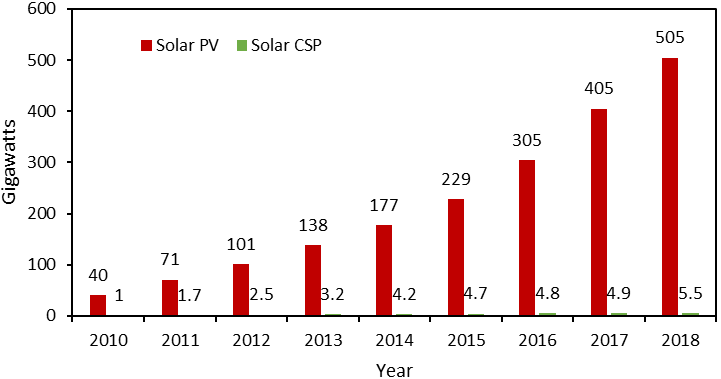
Despite the advantages of solar energy, it hurts the environment. The environmental impact of solar energy started in the seventies [11] [12]. Neff [13] conducted one of the earliest studies about the environmental impacts of PV plants where he studied the adverse impact on land, climate, and emissions. Later, life cycle assessment techniques were used to analyze the environmental impact of PV [14] [15] [16] [17] [18] [19] [20] [21] [22]. These assessments take into consideration the type of the plant (ground based, BIPV, PV or CSP), geographical location, production, operation, maintenance, and decommissioning of the entire plant. For example, Lovins [12] touched upon the benefits and harmful effects of energy sources from the source to end-use form. Some studies focused on regional aspects of specific geographical locations (e.g., Serbia; [23]) or the impact on wildlife [24].
In the year 2018, the global wind power market grew by 51 GW to reach the total installed capacity of 591 GW (Figure 2) [10]. Most of the wind turbines in the world (96%) are onshore wind turbines. China is the world leader in wind power with approximately 210 GW installed capacity. The USA is the second-largest then Germany and India (Figure 3) [10].
The power capacity and size of modern wind turbines are becoming larger with time. Large wind turbines have a larger swept area, they are powerful, and can reduce the cost of the generated renewable power. The modern wind turbines are rated between 0.5 and 2 MW with a rotor diameter ranges from 40 to 90 m [25].
The global wind energy (installed GW)
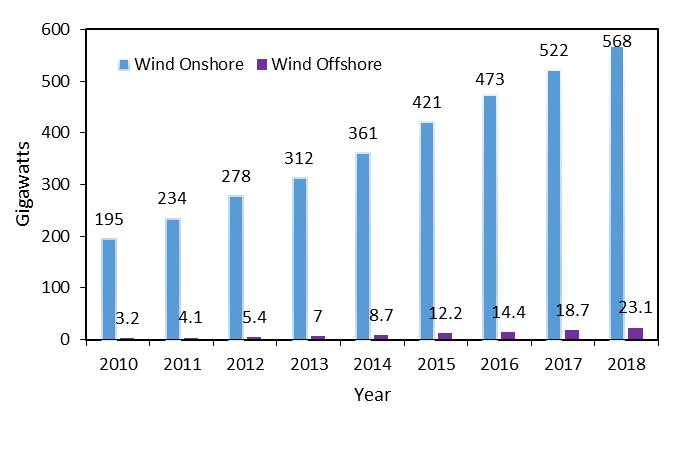
Rapidly falling costs per kilowatt-hour has made both solar and wind energy an attractive option for new power generating capacity in many markets around the world. China is a world leader in PV installations (Figure 3). 37% of the worlds' wind installations and 35% of the world's solar installations are in China [10].
Wind and solar energies - the top six countries
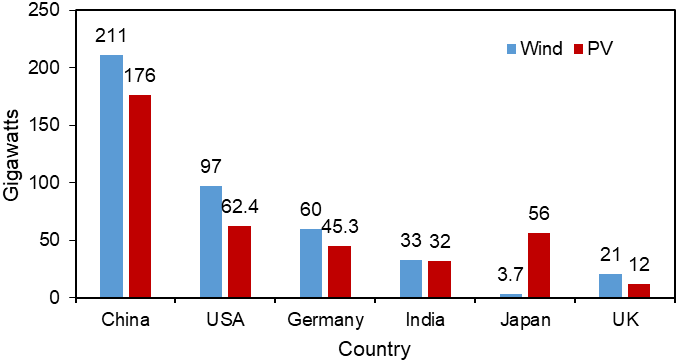
Despite that wind power is environmentally benign, it still has some impact on human life [26]. There are a significant number of life cycle analyses of the environmental impacts of wind turbines, with considerable inconsistencies due to site selection or system size. For example, Raadal et al. [27] [28] showed that onshore wind turbines with a capacity between 30 kW and 3MW can generate total emissions of 4.6–55.6g CO2 /kWh. In another study conducted by Lenzen and Munksgaard [29], higher values of greenhouse gas emissions (7.9 to 123.7g CO2 /kWh) are reported for onshore wind turbines with a capacity between 0.3 kW and 3 MW.
All power production facilities affect the environment and the ecosystems on Earth. Solar and wind systems are raising in various regions in the world and are no longer specific anymore to coastal, mountainous, desert or arid regions. Thus the importance of studying the environmental impact essential for the sustainability of these systems. This impact on the environment varies according to the system type and its geographical location on the planet.
The disagreement of the results of the life cycle analyses in the literature is caused mainly by the inconsistencies of the used parameters, such as solar radiation or wind conditions, site selection, and installation sizes. Some modeling approaches use different pathways when evaluating the same environmental impact which may affect the result of life cycle analysis [30]. Overall, such an inconsistency in life cycle analysis results limits the decision-making process, since it prevents explicit determination of the environmental impact of certain wind or solar installations. This study provides a detailed description of the solar and wind installations' environmental impact on flora and fauna. This description may help in conducting a detailed life cycle analysis and a sophisticated approach, which may lead to practical methods and successful implementation of sustainable solar and wind power production.
Both wind and solar systems have shown significant growth in the last years, they also have a significant impact on the environment, such as noise, biodiversity impact, visual and climatic impact. Although the above-mentioned impacts seem insignificant when compared with traditional fuels, their impact on the whole ecosystem shouldn’t be ignored. It is important to investigate these negative impacts, especially the potential of long-term impact in order to come up with intelligent solutions to maintain the sustainability of solar and wind energy.
This review was conducted by searching several scientific publishers' websites, research databases and the internet using the following search-terms: solar and environmental impact, solar and land, solar and water, solar and biodiversity, solar and visual, solar and noise, solar and health, solar and microclimate, wind and environmental impact, wind and land, wind and water, wind and biodiversity, wind and visual, wind and noise, wind and health, wind and microclimate.
Both concentrated solar thermal and photovoltaic solar plants have an impact on the environment. This impact varies depending on the size and type of the solar plants. This section reviews the environmental impact of solar energy on biodiversity, water consumption, land use, land cover, noise, glare, climate and health.
The integration of small-scale distributed installations or large scale solar plants into the built environment will likely have a negligible direct impact on biodiversity [31]. In this section, the impact of both CSP and PV plants on undisturbed habitats will be the focus of the discussion.
Many studies quantifying the direct impact of large scale solar projects on biodiversity [24] [32] [33] [34]35]. During construction, installation, and operation of both PV and CSP, the soil is graded and in some cases, herbicides are used to eradicate the vegetation. The graded soil and new roads can further increase organisms' mortality rates and the extinction of native species [36] [37].
In arid or semiarid regions, the desert vegetation controls the transport of sand, dust, and sediments. Removal of vegetation, grading the land and the construction of roads by solar energy infrastructure causes soil loss by storms and floods [38] [39].
Large scale solar energy infrastructures may block the movement as well as seasonal migration of wildlife species which may cause a gene migration disruption between different species [40] [41]. This habitat loss and fragmentation could threaten biodiversity and disrupt the eco-system balance [42] [43].
To date, most solar thermal plants are constructed in arid or semiarid areas, which typically have a unique and fragile soil and habitat. An Environmental assessment statement of solar projects in desert regions southwest of the USA [44] reported that the solar plant would significantly impact and threaten desert tortoise, as well as special-status animals and plant species in the local area. The statement has affirmed the necessity of extensive efforts to mitigate impacts on wildlife and habitat.
Concentrated solar systems could pose a marginal threat to insects, and birds flying nearby the focus of the reflectors. McCrary et al. [45] study the quantitative impact of concentrated solar plants on wildlife, where they calculated the death of birds, bats, and insects. They found that the impact was low compared to other anthropogenic activities.
In the case of PV plants, the occupation of land has a major impact on wildlife and habitat. An environmental impact report prepared for a utility-scale PV project (550 MWp) in central California found that tens of protected species in the region are affected [46]. Also, the shade of PV arrays changes the microclimate, reshaping the vegetation biodiversity.
Besides the direct impact, there are also indirect and regional impacts on biodiversity. The elevated levels of greenhouse gases and nitrogen oxides resulting from the increased vehicle activity or gas burners in concentrated solar plants may promote the growth of invasive grasses that increase fire occurrence frequencies [47] [48]. Additionally, chemicals such as antifreeze agents, herbicides, dust suppressants, rust inhibitors that are used during the maintenance of solar plants may have long term harmful effects of the local and regional biodiversity [24] [49].
To protect solar energy facilities from floods, water channels are established in the vicinity of support structures to facilitate the quick divert of surface water and have it flow away from the facility. This diversion of flow can dramatically affect water availability and desert habitat quantity and quality [50].
Dust. Large scale solar facilities substantially impact the landscape through site preparation, grading, vegetation removal, and construction of roads. All of these activities result in dust and particle emissions, especially in the desert and arid regions [51]. Dust emissions can alter plant fertility and water-retention potential of the soil. Physiologically, dust emissions can adversely impact photosynthetic processes, and water consumption of desert plants [52]. Mobilized dust particles can cause root exposure, the burial of plants, and scratch plants leave and stems. The plant damage caused by dust can indirectly affect nutrients and habitat quantity and quality in an adverse manner.
During plant operation, natural dust emissions reduce heliostat, parabolic trough, and panel efficiency in converting sunlight into power or thermal heat. To overcome this challenge, solar plants use various dust suppressants on mirrors and panels. These suppressants can drastically impact the soil, hydrology of the region, and the surface water runoff dynamics [53] [54] [55].
Water is a crucial component of power production systems. [61]. Solar power installations vary in their water use. PV systems need a small amount of water (0.02 m3/MWh), for panel cleaning and dust suppressions in deserts or places rich with dust [62]. While in thermal CSP systems, the majority of the water (85-95%) is used in the cooling cycle and only a small amount of water is used in producing the steam.
Arid and semiarid regions are blessed with high solar irradiance and are suitable for large scale solar projects [63]. However, they are water-constrained areas and water use by solar plants may cause conflict and create competition on the water for agricultural and domestic needs [64] [65].
The water consumption rates in CSP depend on the cooling techniques; wet, dry, or a hybrid cooling (a combination of both) [65].
Concentrating solar thermal power plants with wet cooling system has a considerably large water footprint and consumes large quantities of water (~3 m3/MWh), which is nearly equivalent to the quantity used in traditional nuclear or coal thermal plants [64] [65] [66] [67]. The solar power plants with wet cooling systems are more profitable and efficient [68] compared to plants that use dry cooling units. It is worthy to mention that the majority of the water used in the cooling cycle is reused, but with lower quality and that may cause pollution to the aquifer.
Water is also used in cleaning heliostats and parabolic troughs to reduce reflectivity losses [69]. The back of the mirrors used for concentrated power systems is coated with silver, the solar radiation passes the reflector twice and thus why CSP systems are more affected by dust compared to PV. The volume of water used for cleaning depends on solar technology and region. Usually, plants installed in desert regions (rich with dust storms) consume more water for cleaning. Although other dust removal technologies (e.g., electrostatic, robot cleaning chemical sprays) exist, most are new, and their environmental impact is not completely understood [64] [70].
Land-use efficiency is a key parameter that determines the effectiveness of land use for energy production. It also defines the plant′s generated power relative to the used area of land (W/m2) by the construction throughout the life cycle of the installation [63] [71]. The energy conversion chain includes raw materials exploration, extraction, processing, manufacture, plant construction, power generation, operation, maintenance, decommissioning, and disposal. Thus, energy production processes highly impact the land. In some cases, the damage caused to the land is irreversible and the land cannot be restored to its original state [71] [72] [73].
Utility-scale renewable energy generation facilities can have substantial footprints in terms of land and water use [22]. Many studies in the literature that investigate land use characteristics.
The type and capacity of large scale solar systems are the main factors impacting land-use efficiency. Hernandez et al. [63] found that the land-use efficiency of large scale solar systems in California, to be 4.6 W/m2 for PV and 11.2 W/m2 for CSP. Fthenakis and Kim [71] found out that the direct land use of PV ranges between 402 and 463 m2/GWh.
The impact of large scale solar systems on land-use and land-cover change is relatively smaller than other energy systems [74]. PV systems require the largest land-use when compared to wind, hydroelectric, and biomass [63] [71] [75].
Zanon and Verones [76] discussed the risk of PV plants on the use of fertile areas or the impact of the construction and operation of the facility on the landscape. The competition between land use for agriculture and energy always attracted the attention of researchers, some studies compared the suitability of land (arable, marginal) for agriculture and energy [77] [78] [79] [80]. Tsoutsos et al. [22] study the impact of large scale solar plants on land use, they point out that PV plants can impact the potentially cultivable land and the power output is therefore considered as competitive with food production, similar to the energy-food competition on land in the case of energy crops production [81].
The amount of solar radiation is the major factor impacting the land needed for solar plants. The higher solar radiation leads to less land use for the same capacity of solar plants [82]. CSP plants require land that should be relatively flat. The land used by CSP includes solar arrays, heliostats or parabolic troughs, substations, thermal storage facilities, access roads, service buildings, and other infrastructure.
The visual impact of PV systems is highly dependent on the nature surrounding the system. In the case of installations near areas with natural beauty, the visual impact can be significantly high. In the case of building integrated systems, it may have a positive aesthetic and educational impact. It can contribute to controlling the shading, lighting, and ventilation [22]. It’s also attractive when used in areas where the public can see it e.g. Parks, where it may serve as a tool for increasing public awareness of these technologies [22].
Usually, CSP plants are installed far away from the public residential areas and have a very minimum visual impact. The visual impact of the solar towers is high compared to parabolic concentrators, Stirling dish engines, and Fresnel plants, due to the height of the tower which may reach up to 240 meters [83].
Solar water heaters both flat panels and evacuated tubes are usually designed to fit closely to the existing roof design. Furthermore, architects use solar collector elements to enhance the aesthetic appeal of a building.
There is almost no noise generated from small and large scale PV power plants. CSP plants are less noisy compared to other renewable and non-renewable power plants, such as wind farms or coal and gas-fired plants. In CSP thermal plants, the steam or gas turbine is located in the middle of the plant and is surrounded by heliostats or parabolic troughs. Thus the turbine noise is unlikely to disturb the neighboring public.
The reflected radiation from the surface of the PV modules, mirrors, or from the receiver in a solar tower can cause a glare that may lead to a temporary reduction or loss of vision.
In hot seasons and due to the infrared component of the solar insolation, the temperature of the photovoltaic panels can reach 70 °C or higher [84]. This increase in PV module temperature reduces the electrical output of the module, heats the air surrounding the system, and influence the microclimate of the site. Also, such changes in temperature may cause a problem in the outdoor built environment and impact the outdoor thermal comfort conditions and generate a heat island effect. The overheating of the PV arrays can be avoided by passive cooling [84].
In the case of concentrating systems, thermal facilities can increase the albedo in deserts by 30%-56%, which could impact local temperatures and precipitation through wind pattern changes and evapotranspiration. Also, large concentrating power plants, specifically the dry-cooled facilities, may generate a significant amount of waste heat that can be transferred by wind creating drought conditions. The heat generated by solar tower facilities, especially at the focal point, can burn birds and insects [22] [32] [85] [86].
In CSP plants, collectors create shading and this may increase or decrease the soil temperature depending on the season and the location [87].
Many chemicals are used in manufacturing PV panels. For example, in thin-film solar cells, carcinogens such as cadmium are used. Similarly, many other toxic chemicals are used to maintain solar panels clean. European Union initially banned the use of cadmium and other heavy metals. However, in 2010, the EU excluded the PV panel from this ban in order to attain the set targets for renewable power generation [88]. The inappropriate handling in the recycling process of PV modules can expose the public to toxic materials such as cadmium, arsenic, and silica dust [89] [90] [91].
In CSP systems, the heat transfer oil is carried by long pipelines that connect the long rows of mirrors, dishes, or parabolic troughs. Some solar thermal facilities have thermal storage units that use molten salts as a heat storage medium which may leak into the surrounding environment [22] [24].
Large scale concentrated solar facilities, especially wet-cooled solar facilities, use toxic chemicals in their cooling and recirculating systems, such as antifreeze agents, rust inhibitors, acids, alkali, heavy metals, and herbicides [22] [49].
During the construction of large scale solar projects, hazards like soil-borne pathogens [92] and different sizes of particulate matter [93] [94] are released. These hazards can lower the local air quality and impact public health [95].
Generally, there is no direct air or soil pollution caused by wind energy. Only a negligible amount of carbon emissions is produced during the building and maintenance processes. However, this amount of CO2 is very small compared to other traditional non-renewable power plants. It is also important to mention that wind energy does not produce gas emissions such as CO2, SOx, mercury, particulate matters, or other types of air pollutants [96]. An Irish study estimates that reductions in CO2 emissions can range from 0.33 to 0.59 tons of CO2 per MWh [97].
Assessment of the potential effects of any proposed energy project on the local flora and fauna is essential for any environmental assessment. Bird disturbance is known as a major issue for wind energy development, particularly for remote sites with rare habitats. The direct impact of wind energy is the death caused by the collisions of birds with wind energy plants. Birds are considered the largest victim groups in mortality caused by collision with wind turbines around the world [98]. However, deforestation and urban expansion can cause much higher bird fatalities [24]. Sovacool [99] argues that wind turbines kill about 95% fewer birds compared to fossil fuels. For example, for a 1GW wind power plant, the annual birds killed, is only 20, while 2000 were killed by vehicles and high voltage transmission lines, and 1500 were killed caused by hunting [100]. Some studies indicate that birds can quickly adapt to newly installed wind turbines [101].
The indirect wind energy impacts are habitat disruption, avoidance, and displacement [102]. For example, sea creatures are impacted by offshore wind turbines. Thomsen et al. [103] reported that dab and salmon's behaviors can be influenced by the perceived pile-driving pulses caused by wind turbines construction and operation.
Factors affecting birds' collision with wind turbines. There are several factors that lead to a collision of the birds with wind turbines [104]
Flashlights. The light emitted from wind towers attracts birds especially during foggy nights and poor weather conditions. Thus, birds fly toward the wind turbines and this increases collision possibilities with wind towers and blades [105].
Weather conditions. Gregory et al. [106] point out that 3 out of 48 bird mortalities happen when the weather is not a factor. Migrating birds tend to fly at lower altitudes during bad weather such as storms, low clouds, and rain. This increases birds' collision potential with wind turbines.
Tower design. Old wind turbines have a small capacity, low hub heights, and shorter blades. Old wind farms usually have a large number of small turbines. The low height of the turbines attracts birds' nesting and the blades' high rotation speed increase the bird mortalities [102]. Josimovic [107] shows that high wind generators' (145m) do not present a risk for the migrating birds since they fly at much higher altitude.
Noise pollution is considered a critical environmental impact of wind farms. The wind turbine noise can be a major annoyance to people [108] [109]. The value of properties nearby the wind farms may be negatively impacted by the noise greeted by wind turbines.
There are two types of noise emitted by wind turbines; mechanical and aerodynamic noise. The noise caused by the moving components such as bearings, gear, and power generator is called mechanical noise. The level of this noise is affected by turbine size, wear and tear, lack of maintenance, and poor component designs [110]. The mechanical noise can be lowered by using proper acoustic insulation of the turbine house and by the use of anti-vibration support footings [111]. The second type of noise is the aerodynamic noise which is caused by the dynamics of how the airflow and passes the blades of the turbine. The interaction of blades with turbulent wind causes the "whooshing" sound. In general, the aerodynamic noise increases by the increase of the blade tip speed [112]. The reduction of the aerodynamic noise can be achieved by proper blades design [111]. The noise of the blade is less with slow blade tip speeds and with large wind turbines [113].
Some researchers have done research and surveys on the impact of aerodynamic noise's low frequency on people and have found that it may disturb people who live close to wind turbines. Miller and Keith [113] point out that sound pressure levels may cause anxiety indications such as headaches to those who live close to the wind turbines. They also mention that wind turbines' low-frequency aerodynamic noise can cause sleep disorders and hearing problems. However, Leung and Yang [25] point out that having obstacles in the surrounding lines of the wind farm may decrease the noise pollution.
Pedersen [114] has studied wind turbines’ sound pressure impact on peoples’ well-being, showing that those who were disturbed significantly by wind turbines suffer from stress symptoms and headaches. Punch et al. [115] have reviewed and summarized the literature in this field and concluded that wind turbines' low-frequency aerodynamic noise may cause sleeping and hearing problems, and may damage the vestibular system. However, there are suggested possible solutions to solve this issue. For example, some suggested house structure changes to reduce or even eliminate the noise or to build the wind farm at 2 km distance from where they live [109]. Also, optimizations made on blade design [116] can reduce the level of noise by 0.5 to 3.2 dB.
The visual impact of wind turbines is another critical factor that influences the public perception of this technology. However, among all environmental impacts of wind turbines, measuring and estimating the visual impact is a challenging task [101]. People are subjective when they evaluate the visual impact; some individuals enjoy looking at wind farms, while some people have contrasting views. Visual impact surveys conducted in the UK have shown that the majority (70%) of people doesn’t oppose wind turbines [117]. While some surveys point out that people from the tourism industry believe that wind farms may negatively impact local tourism [25].
The wind turbines’ visual impact is affected by several factors, e.g. the proximity of the observer to the wind farm, size, type, color or contrast, number of wind turbines, wholly or partially seen, the number and rotational speed of blades, the arrangement of the turbines across the site, the local lighting conditions (shadow flickering) operation time and local turbine history [118] [100].
Carefully planned wind farms can fit well into and be part of the landscape. For example, Josimovic [107], argues that visual impact was not a major problem for Bavaniste wind farm in Serbia, and they argue that the farm will act as a catalyst in developing the local tourism, which contradicts the observation of Leung and Yang [25].
As the wind turbines become larger in size and number, there are some speculations that they could affect the local climate. In the latest studies of Chapman [119] and Miller and Keith [113] they argue that wind power can impact the local climate by changing the macro-climate at the local level, but its impact on the global average surface temperature would be insignificant.
The land use of wind power depends on the turbines’ spacing and their configuration. The land use includes land preparation, construction, access roads, and transmission lines. Usually, wind turbines use less than 10% of the wind farm areas [120]. Compared to other energy systems, wind energy has a minimal land footprint. However, the visual impact on the landscape may affect the value and uses of neighboring lands.
The share of electricity production in the world greenhouse gas emissions is 40%. In 2016, almost 64.5% of world electricity was generated by fossil fuel-powered plants. The environmental impact of future electricity production has been the focus of debate among general public and policymakers [121].
Societies' increased environmental awareness and their growing concerns about climate change have required a full understanding of the potential environmental impacts of electricity production worldwide. Therein lies a fragile balance of large scale renewable energy developments: on one hand it is necessary to mitigate climate change impacts and help protect the environment, on the other hand, if not carefully planned, it could significantly cause and add damage to the one already caused by fossil fuels.
This study sheds light on the visual, noise, and water use impacts of wind and solar energy and their implications on biodiversity, land, surface and underground water, and human health.
The transition to the electricity sector with a low carbon footprint could result in either a higher or lower water footprint, depending on the used technology and cooling technique employed. Table 1 shows the water consumption for electricity production by different energy systems. As seen from the table, non-thermal renewable technologies, such as PV and wind, in addition to producing clean and abundant energy, they consume the least amount of water compared to other renewable and fossil fuel energy sources. However, CSP systems with wet cooling techniques use almost similar values as coal.
Water consumption for different energy systems
Energy System |
(L/MWh) |
References |
PV and CPV |
0-20 (for cleaning) |
|
CSP with wet cooling |
2900-3800 |
|
CSP with dry cooling |
250-300 |
|
Wind |
0-4 |
[122] |
Geothermal |
5300 |
[61] |
6800 |
[128] |
|
230-6800 |
[120] |
|
Coal |
1800-4200 |
|
Nuclear |
1500-3200 |
Table 2 and Table 3 are documented greenhouse gas emissions and land-use intensity for different energy technologies.
Comparison of producing greenhouse gas emissions for different energy generation systems.
Energy system |
g CO 2 /kWh |
References |
Coal |
975 |
[132] |
1004 |
[133] |
|
960-150 |
[134] |
|
Gas |
608 |
[132] |
543 |
[133] |
|
Oil |
742 |
[135] |
778 |
[134] |
|
Nuclear |
24 |
[135] |
66 |
[134] |
|
CSP Parabolic trough |
26 |
[136] |
13 |
[134] |
|
CSP Power tower |
38 |
[136] |
PV Mono Crystalline Silicon |
45 |
[137] |
91 |
[138] |
|
60 |
[139] |
|
44 |
[140] |
|
61 |
[138] |
|
41 |
[141] |
|
PV poly Crystalline Silicon |
53.4 |
[142] |
26.4 |
[19] |
|
72.4 |
[143] |
|
9-12 |
[138] |
|
32 |
[144] |
|
PV Thin Film Amorphous Silicon |
21 |
[147] |
34.3 |
[145] |
|
15.6-16.5 |
[138] |
|
39 |
[146] |
|
50 |
[147] |
|
PV Thin-film cadmium telluride |
14 |
[145] |
23.6 |
[20] |
|
25 |
[144] |
|
48 |
[20] |
|
Copper indium gallium diselenide |
95 |
[20] |
10.5 |
[138] |
|
21 |
[148] |
|
46 |
[149] |
|
Wind |
9.7-16.5 |
[150] |
8.2 |
[151] |
|
17.5 |
[27] |
|
7.9-23.7 |
[29] |
|
Hydro |
10-13 |
[134] |
Geothermal |
38 |
[134] |
As seen from Table 2, the gas emissions for various types of PV, CSP and wind energy are significantly lower than emissions generated by fossil fuels.
Land use intensity for different energy systems
System |
Land use m 2 /MWh |
References |
Parabolic trough |
0.366 |
[62] |
0.3322 |
[152] |
|
15 |
[153] |
|
Wind |
72 |
[91] |
1 |
[153] |
|
PV |
28–64 |
[154] |
Geothermal |
18–74 |
[155] |
2.5 |
[153] |
|
Coal |
0.2-5 |
[153] |
As seen from tables 2 and 3, wind energy and solar PV systems show substantial inconsistencies in the values of land use and greenhouse gas emissions intensities. This inconsistency is caused by many factors; raw material extraction, manufacturing, location of the project, the size of the installation, and the life expectancy of the project.
Solar and wind resources are directly connected to the geographic location. Essentially, the amount of power generated from the solar and wind installation is based on the resource, the higher the generated power from the same power plant leads to lower GHG emissions and land use intensities. For example, the generated power from a wind turbine will vary according to the landscape topography of the site, as mountains, water bodies, buildings, and even trees can affect the wind speed. Similarly, the location of the solar installation has the same effect on the generated power from the PV plant; solar radiation varies across the globe and on an hourly and seasonal basis. The type of built environment and shading can thus play a role in solar installation’s performance.
The size of the installation also affects the greenhouse gas emissions, the large and high capacity wind turbines and solar installations, generate significantly less greenhouse gas emissions generated per unit of electricity. Zimmermann and Gößling-Reisemanna [156] found that, at the same site, higher turbines receive higher wind speed and generate more power which then leads to less greenhouse gas emissions. Kabir et al. [157] calculated the greenhouse gas emission intensity for three different wind farms with a similar total capacity 20 wind turbines, each 5 kW, 5 wind turbines 20 kW, one wind turbine of 100 kW). They found that the one 100 kW wind turbine generates only one-third of the emissions that are generated by the smallest farm.
Solar energy also follows the sizing advantage of wind energy, Nunget and Sovacool [158] shows that the greenhouse gas emissions are noticeably lower for larger PV installations.
The life expectancy of solar and wind energy systems has a significant impact on greenhouse gas emissions. As noted in this article, the majority of greenhouse gasses for PV and wind are generated during the extraction and manufacturing and installation process. Thus the longer life expectancy the lower greenhouse gas emissions through the whole life of the system. Nugent and Sovacool [158] show that a wind system with a 20-year life expectancy results in an average of 40.7 g CO2/kWh, and 30-years reduces it to 25.3 g CO2/kWh.
To minimize the impact of the large scale solar projects on a certain region, locating the project on land where the impact of flora and fauna is relatively small can be a good practice that leads to both clean energy production and conservation goals [34] [159]. For instance, Fluri [159] pointed out that right and strategic site selection of a large scale concentrated solar project in South Africa has assisted in preventing negative impact on endangered or vulnerable vegetation. Another strategy to reduce the impact of large scale solar projects is to employ translocation programs, to transfer the impacted native species in other reserve areas. The success rate of translocation programs is low (e.g., <20% [160] [161]), but these programs can be useful when other mitigation options are unfeasible [162]. These mitigation techniques to minimize biodiversity impacts are costly, do not target all species and are not efficient to support the relocation of the targeted organisms [24]. Additionally, relocating birds is not possible and birds and certain components of large scale solar projects could attract birds to nest in them.
One benefit of large scale solar plants on biodiversity is the allocation of funds for research and development. In many countries, utility-scale solar plants’ developers provide financial support for environmental impact mitigation activities throughout the lifetime of the power plant, these funds, when used properly, can be a benefit to local wildlife [163]. In the US, for example, the DOE requires and extensive monitoring of wildlife and habitat restoration [164] on solar plants' sites. These monitoring and restoration activities can eliminate invasive or overpopulating species and can protect endemic species.
To reduce water consumption rates of CSP, the dry cooling technique can be used and may save up to 80% to 95% of the water use [66] [165] this option is a viable one in water-limited ecosystems such as arid and semiarid regions. It is worthy to mention that dry cooling is less efficient and the initial setup cost is higher [54]. But the long-term water-saving costs can offset dry cooling costs by 87–227% [66] [166]. On the other hand, facilities that utilize dry-cooling, use air to cool the steam and in order to achieve higher cooling efficiencies, a larger land is required which may impact wildlife habitats. Moreover, technologies with dry cooling become less efficient in regions with temperatures higher than 38 °C [167]. Additionally, the gas emissions from power plants with dry cooling are higher (5% to 7%) compared to wet cooling power plants. This is due to the low efficiency caused by the power consumed by the cooling fans.
In regard to wind energy, there are several techniques to minimize the environmental impact and thus increase the wide use of wind technology. Wildlife surveys can be conducted during the impact assessment of wind farm installations to understand the birds’ breeding and feeding dynamics. These surveys can help in minimizing the threats to birds [168]. There are several design aspects and developments to reduce the risk to migratory birds, e.g. the use of larger and more visible blades, low rotational speed, the use of tubular towers with a smooth exterior, internal ladders and underground wiring prevents the roosting and nesting of birds on the tower and reduces the presence of birds in wind farms [169].
One of the techniques used to reduce wind turbines' visual impact, is to paint them in grey to make them blend with the skyline color [170] [171]. Ian and David [172] report that the impact of the wind turbine's contrast level increases with the increase of contrast with the surrounding environment and decreases with distance.
A shadow flickering is another important impact of wind turbines; this phenomenon is caused when rotating blades cast shadows through the windows of neighboring houses. The scale of this phenomenon depends on wind speed and the sun position in the sky. This phenomenon worsens at sunset and sunrise or during winter seasons, or at faster wind velocities. The use of light sensors may help in minimizing the shadow flickering effect. These sensors help in shutting down the turbines when shadow flickering may happen. In addition to flickering, the light reflection of the turbine body (tower and blades) may cause a problem to neighboring properties. This problem can be eliminated by optimizing the smoothness of the blades' surface as well as by using mat paintings [171].
Wind and solar energy, especially towers, will continue to disturb birds and will continue to challenge energy developers, particularly for remote sites with endangered and rare species. To protect bird and minimize collision with towers and turbines, avian radars can be used to detect birds in the area. In case the birds are approaching, the wind farm and there is a real threat to them the systems automatically shut down the wind turbines [169].
Proper planning and attention should be paid to all beneficiaries of wind and solar installations. Lowering the impact of solar and wind energies on the flora and fauna of an area is possible only by conducting a comprehensive environmental assessment that takes into consideration all factors mentioned in this article.
This study reviewed and evaluated the environmental impacts of solar and wind energy, which is conceptually a multi- and interdisciplinary field. Better planning and understanding of solar and wind installations, can minimize and may prevent the above mentioned and foreseeable negative impacts. As societies move toward a carbon-free economy, the combination of solar and wind energy with emerging energy storage and carbon capture and sequestration technologies is unavoidable. This successful combination with energy storage techniques will significantly accelerate the solar and wind energy development. This combination will also increase the importance of environmental impact analysis at both national and global levels. The complexity and inter-disciplinary of the environmental field impact requires proper optimization, well-organized coordination, and research to further advance and maximize the socioeconomic and environmental benefits of large scale wind and solar energy systems.
2017 , 2017
, , 2017
, Analysis of renewable energy incentives in the Latin America and Caribbean region: The feed-in tariff case , 2013, https://doi.org/10.1016/j.enpol.2012.09.024
, Is renewable energy effective in promoting growth? Energy Policy 2012;46:434-42, https://doi.org/10.1016/j.enpol.2012.04.006 , 2012, https://doi.org/10.1016/j.enpol.2012.04.006
, The politics of renewable energy policies: The case of feed-in tariffs in Ontario Canada , 2013, https://doi.org/10.1016/j.enpol.2013.01.009
, The impact of wind generation on electricity spot-market price level and variance: the Texas experience , 2011, https://doi.org/10.1016/j.enpol.2011.03.084
, Environmental impacts of utility-scale solar energy , 2014, https://doi.org/10.1016/j.rser.2013.08.041
, Prepared by Working Group III of the Intergovernmental Panel on Climate Change [O , 2011
, The potential for avoided emissions from photovoltaic electricity in the United States , 2012, https://doi.org/10.1016/j.energy.2012.08.025
, , 2019
, Energy technologies and natural environments: the search for compatability , 1978, https://doi.org/10.1146/annurev.eg.03.110178.000533
, Energy strategy: the road not taken? Foreign Affairs 1976;55: 65-96, https://doi.org/10.2307/20039628 , 1976, https://doi.org/10.2307/20039628
, The social cost of solar energy , 1981
, Life-cycle analysis of renewable energy systems , 1994, https://doi.org/10.1016/0960-1481(94)90161-9
, Environmental impacts of PV systems: ground based vs , 1997
, The environmental impact of photovoltaic technology , 1998
, Life-cycle assessment of photovoltaic systems: results of Swiss studies on energy chains , 1998, https://doi.org/10.1002/(SICI)1099-159X(199803/04)6:2
, Simplified life-cycle analysis of PV systems in buildings: present situation and future trends , 1998, https://doi.org/10.1002/(SICI)1099-159X(199803/04)6:2
, Parameters affecting the life cycle performance of PV technologies and systems , 2007, https://doi.org/10.1016/j.enpol.2006.10.003
, Life cycle assessment and energy pay-back time of advanced photovoltaic modules: CdTe and CIS compared to poly-Si , 2007, https://doi.org/10.1016/j.energy.2006.10.003
, Life cycle assessment of photovoltaic electricity generation , 2008, https://doi.org/10.1016/j.energy.2007.11.012
, Environmental impacts from the solar energy technologies , 2005, https://doi.org/10.1016/S0301-4215(03)00241-6
, A review of concentrating solar power plants in the world and their potential in Serbia , 2012, https://doi.org/10.1016/j.rser.2012.03.042
, Wildlife conservation and solar energy development in the desert southwest, United States , 2011, https://doi.org/10.1525/bio.2011.61.12.8
, Leung, Y , 2012, https://doi.org/10.1016/j.rser.2011.09.024
, A review on Environmental Kuznets Curve hypothesis using bibliometric and meta-analysis , 2018, https://doi.org/10.1016/j.scitotenv.2018.08.276
, Life cycle greenhouse gas (GHG) emissions from the generation of wind and hydro power , 2011, https://doi.org/10.1016/j.rser.2011.05.001
, GHG emissions and energy performance of offshore wind power , 2014, https://doi.org/10.1016/j.renene.2013.11.075
, Energy and CO2 life-cycle analyses of wind turbines - review and applications , 2002, https://doi.org/10.1016/S0960-1481(01)00145-8
, LCA discussions comparison of three LCIA methods comparison of three different LCIA methods: EDIP97, CML2001 and eco-indicator 99 does it matter which one you choose? Int J LCA 2003;8:191-200, https://doi.org/10.1007/BF02978471 , 2003, https://doi.org/10.1007/BF02978471
, The land use-climate change-energy nexus , 2011, https://doi.org/10.1007/s10980-011-9606-2
, Avian mortality at a solar energy power plant , 1986
, Powerline corridors as possible barriers to the movement of small mammals , 1977, https://doi.org/10.2307/2425117
, An Approach to Enhance the Conservation-Compatibility of Solar Energy Development , 2012, https://doi.org/10.1371/journal.pone.0038437
, The impact of a utility corridor on terrestrial gastropod biodiversity , 2012, https://doi.org/10.1007/s10531-011-0216-8
, Roads as conduits for exotic plant invasions in a semiarid landscape , 2003, https://doi.org/10.1046/j.1523-1739.2003.01408.x
, The effect of a disturbance corridor on an ecological reserve , 1995, https://doi.org/10.1111/j.1526-100X.1995.tb00098.x
, Aeolian and fluvial processes in dryland regions: the need for integrated studies , 2011, https://doi.org/10.1002/eco.258
, The ecology of dust , 2010, https://doi.org/10.1890/090050
, DOE/EA-1663, Environmental assessment for BP solar array project Brookhaven National Laboratory Upton, New York; 2009. , 2009
, Biological consequences of ecosystem fragmentation: a review , 1991, https://doi.org/10.1111/j.1523-1739.1991.tb00384.x
, Effects of habitat fragmentation on biodiversity , 2003, https://doi.org/10.1146/annurev.ecolsys.34.011802.132419
, Quantifying threats to imperiled species in the United States , 1998, https://doi.org/10.2307/1313420
, Final staff assessment and draft environmental impact statement and draft California Desert conservation area plan amendment: Ivanpah solar electric generating system , 2010
, Wildlife interactions at solar one: final report , 1984
, San Luis Obispo: County of San Luis Obispo Planning Department; 2010. , 2010
, Impacts of atmospheric nitrogen deposition on vegetation and soils at Joshua Tree National Park , 2009
, Risk-based determination of critical nitrogen deposition loads for fire spread in southern California deserts , 2010, https://doi.org/10.1890/09-0398.1
, The likely adverse environmental impacts of renewable energy sources , 2000, https://doi.org/10.1016/S0306-2619(99)00077-X
, 1989 , 1989, https://doi.org/10.2136/sssaj1989.03615995005300050045x
, Responses of wind erosion to climate-induced vegetation changes on the Colorado Plateau , 2011, https://doi.org/10.1073/pnas.1014947108
, Surface dust impacts on gas exchange in Mojave Desert shrubs , 1997, https://doi.org/10.2307/2405275
, Hydrologic impacts of disturbed lands treated with dust suppressants , 2003, https://doi.org/10.1061/(ASCE)1084-0699(2003)8:5(278)
, 2013 , 2013
, Siting solar energy development to minimize biological impacts , 2013, https://doi.org/10.1016/j.renene.2013.01.055
, HH , 1977, https://doi.org/10.2307/2424698
, A regional landscape approach to maintain diversity , 1983, https://doi.org/10.2307/1309350
, Diversity of small mammals in a powerline right-of-way and adjacent forest in east Tennessee , 1979, https://doi.org/10.2307/2424918
, Fragmentation of habitat by roads and utility corridors: a review , 1990, https://doi.org/10.7882/AZ.1990.005
, Plant response to utility right of way construction in the Mojave Desert , 1980, https://doi.org/10.1007/BF01866455
, Energy demands on water resources: report to congress on the interdependency of energy and water , 2006
, Life-cycle uses of water in U.S , 2010, https://doi.org/10.1016/j.rser.2010.03.008
, in review, The land-use efficiency of big solar , 2014, https://doi.org/10.1021/es4043726
, 2009 , 2009
, Enhancement of a stand-alone photovoltaic system′s performance: reduction of soft and hard shading , 2011, https://doi.org/10.1016/j.renene.2010.09.018
, Life cycle assessment of a parabolic trough concentrating solar power plant and the impacts of key design alternatives , 2011, https://doi.org/10.1021/es1033266
, Energy's tricky tradeoffs , 2010, https://doi.org/10.1126/science.329.5993.786
, 2003 , 2003, https://doi.org/10.2172/15005918
, Impact of dust on solar photovoltaic (PV) performance: research status, challenges and recommendations , 2010, https://doi.org/10.1016/j.rser.2010.07.065
, Review of self-cleaning method for solar cell array , 2011, https://doi.org/10.1016/j.proeng.2011.08.1135
, Land use and electricity generation: a life-cycle analysis , 2009, https://doi.org/10.1016/j.rser.2008.09.017
, Evidence of a regional subsidence and associated interior wetland loss induced by hydrocarbon production, Gulf Coast region , 2006, https://doi.org/10.1007/s00254-006-0207-3
, Life cycle greenhouse gas emissions of crystalline silicon photovoltaic electricity generation , 2012, https://doi.org/10.1111/j.1530-9290.2011.00439.x
, Energy sprawl or energy efficiency: climate policy impacts on natural habitat for the United States of America , 2009, https://doi.org/10.1371/journal.pone.0006802
, Syste'mes e'nerge'tiques , 2003
, Climate change, urban energy and planning practices: italian experiences of innovation in land management tools , 2013, https://doi.org/10.1016/j.landusepol.2012.11.009
, Renewable energy and food supply: will there be enough land? Renew , 2005, https://doi.org/10.1016/j.rser.2004.02.003
, Assessment of the renewable energy-mix and land use trade-off at a regional level: a case study for the Kujawsko-Pomorskie , 2013, https://doi.org/10.1016/j.landusepol.2013.05.018
, More solar farms or more bioenergy crops? Mapping and assessing potential land-use conflicts among renewable energy technologies in eastern Ontario, Canada , 2015, https://doi.org/10.1016/j.apgeog.2014.11.028
, 2011 , 2011, https://doi.org/10.1016/j.renene.2011.03.005
, The food v , 2009, https://doi.org/10.1016/j.renene.2008.08.015
, Environment impact of a concentrated solar power plant , 2019, https://doi.org/10.31803/tg-20180911085644
, https://www.ge.com/content/dam/gepower-renewables/global/en_US/downloads/brochures/solar-csp-ashalim-gea32278.pdf [Accessed 29 July 2020] , 2020
, Passive cooling of building-integrated photovoltaics in desert conditions: Experiment and modeling , 2019, https://doi.org/10.1016/j.energy.2018.12.153
, 1994 , 1994, https://doi.org/10.2307/1312281
, The American West at Risk: Science, Myths, and Politics of Land Abuse and Recovery , 2008, https://doi.org/10.1093/oso/9780195142051.001.0001
, Environmental impacts of large scale CSP plants in northwestern China, Environmental Science: Processes and Impacts 2014;16:2432-41, https://doi.org/10.1039/C4EM00235K , 2014, https://doi.org/10.1039/C4EM00235K
, Solar panels win reprieve in EU toxic substance ban , 2011
, Manufacture of amorphous silicon and GaAs thin film solar cells: an identification of potential health and safety hazards , 1984, https://doi.org/10.1016/0379-6787(84)90091-7
, End-of-life management and recycling of PV modules , 2000, https://doi.org/10.1016/S0301-4215(00)00091-4
, Life-cycle assessment of electricity generation options: the status of research in year 2001 , 2001, https://doi.org/10.1016/S0301-4215(02)00088-5
, Soil: a public health threat or savior? Critical Reviews in Environmental Science and Technology 2009;39 (5):416-32, https://doi.org/10.1080/10643380701664748 , 2009, https://doi.org/10.1080/10643380701664748
, Dust in the wind: long range transport of dust in the atmosphere and its implications for global public and ecosystem health , 2001, https://doi.org/10.1023/A:1011910224374
, A focus on particulate matter and health , 2009, https://doi.org/10.1021/es9005459
, Solar energy construction impacts , 2020
, Life cycle energy and greenhouse emissions analysis of wind turbines and the effect of size on energy yield , 2009, https://doi.org/10.1016/j.rser.2009.07.008
, Environmental impact of wind energy, Renew , 2011, https://doi.org/10.1016/j.rser.2011.02.024
, Assessing the impacts of the wind farms on birds , 2006, https://doi.org/10.1111/j.1474-919X.2006.00516.x
, Contextualizing avian mortality: a preliminary appraisal of bird and bat fatalities from wind, fossil-fuel, and nuclear electricity , 2009, https://doi.org/10.1016/j.enpol.2009.02.011
, J Visual impact evaluation in wind farms , 2009
, Wind turbines , 2000
, Footprints in the wind?: environmental impacts of wind power development , 2003, https://doi.org/10.1016/S0140-6701(03)81828-3
, Effects of offshore wind farm noise on marine mammals and fish , 2006
, Assessing impacts of wind-energy development on nocturnally active birds and bats: a guidance document , 2007, https://doi.org/10.2193/2007-270
, Predicting the trends of vertebrate species richness as a response to wind farms installation in mountain ecosystems of northwest Portugal , 2010, https://doi.org/10.1016/j.ecolind.2009.04.014
, avian monitoring studies at the Buffalo Ridge, Minnesota wind resource area: results of a 4-year study , 2000
, The strategic environmental impact assessment of electric wind energy plants: Case study 'Bavanište' (Serbia) , 2010, https://doi.org/10.1016/j.renene.2009.12.005
, Annoyance caused by amplitude modulation of wind turbine noise , 2011, https://doi.org/10.3397/1.3531797
, A quiet revolution , 2009, https://doi.org/10.1049/et.2009.1709
, Noise pollution from wind turbine, living with amplitude modulation, lower frequency emissions and sleep deprivation , 2007
, Wind developments: technical and social impact considerations , 2007
, Wind turbine noise , 1991
, Climatic impacts of wind power , 2018, https://doi.org/10.1016/j.joule.2018.09.009
, Health aspects associated with wind turbine noise , 2011, https://doi.org/10.3397/1.3533898
, Wind-turbine noise: what audiologists should know , 2010
, Reduction of wind turbine noise using optimized airfoils and trailing-edge serrations , 2008, https://doi.org/10.2514/6.2008-2819
, Wind farms are not only beautiful, they're absolutely necessary , 2008
, Visual impact assessment of offshore wind farms and prior experience , 2009, https://doi.org/10.1016/j.apenergy.2008.05.005
, Climatic and human impact on the environment: a question of scale , 2018, https://doi.org/10.1016/j.quaint.2017.08.010
, From LCAs to simplified models: a generic methodology applied to wind power electricity , 2013, https://doi.org/10.1021/es303435e
, Fuel from the Sky, Solar Power's Potential for Western Energy Supply , 2002
, Economic, energy and environmental benefits of concentrating solar power in California , 2006
, Renewable energy technology characterizations , 1997
, Nexant parabolic trough solar power plant systems analysis , 2006
, Final report on the operation and maintenance improvement program for concentrating solar power plants , 1999, https://doi.org/10.2172/8378
, Water Use in Parabolic Trough Power Plants: Summary Results from WorleyParsons' analyses, NREL/TP-5500-49468, https://doi.org/10.2172/1001357 , 2010, https://doi.org/10.2172/1001357
, Concentrating Solar Power 2011: Technology, Costs and Markets, Greentech Media Webinar , 2011
, Water in crisis: a guide to the world's fresh water resources , 1993
, Water Use Benchmarks for Thermoelectric Power Generation , 2006
, Water and Sustainability (Volume 2): An Assessment of Water Demand, Supply, and Quality in the U.S.-The Next Half Century , 2002
, Estimating Freshwater Needs to Meet 2025 Electricity Generating Capacity Forecasts , 2025
, LCA of renewable energy for electricity generation systems-a review , 2009, https://doi.org/10.1016/j.rser.2008.08.004
, Assessment of sustainability indicators for renewable energy technologies , 2009, https://doi.org/10.1016/j.rser.2008.03.008
, Valuing the greenhouse gas emissions from nuclear power: a critical survey , 2008, https://doi.org/10.1016/j.enpol.2008.04.017
, Life cycle greenhouse gas emissions of trough and tower concentrating solar power electricity generation , 2012, https://doi.org/10.1111/j.1530-9290.2012.00474.x
, Effect of shadow and dust on the performance of silicon solar cell , 2011
, An evaluation on the life cycle of photovoltaic energy system considering production energy of off-grade silicon , 1997, https://doi.org/10.1016/S0927-0248(97)00029-9
, Hidden energy and correlated environmental characteristics of P.V , 1992, https://doi.org/10.1016/0960-1481(92)90101-8
, Life cycle assessment of a mediumsized photovoltaic facility at a high latitude location , 2006, https://doi.org/10.1243/09576509JPE253
, The real environmental impacts of crystalline silicon PV modules: an analysis based on up-to-date manufacture data , 2005
, Life cycle GHG emission analysis of power generation systems- Japanese case , 2005, https://doi.org/10.1016/j.energy.2004.07.020
, Evaluation of technical improvements of photovoltaic systems through life cycle assessment methodology , 2005, https://doi.org/10.1016/j.energy.2004.07.011
, Environmental impacts of PV electricity generation-a critical comparison of energy supply options , 2006
, The effect of soiling on large grid-connected photovoltaic systems in California and the Southwest Region of the United States , 2006, https://doi.org/10.1109/WCPEC.2006.279690
, Life cycle assessment of electricity generation systems and applications for climate change policy analysis , 2002
, Energy payback time and CO2 emissions of PV systems , 2000, https://doi.org/10.1002/(SICI)1099-159X(200001/02)8:1
, In: Proceedings of Symposium G-Life Cycle Analysis, Materials Research Society Fall Meeting , 2005, https://doi.org/10.1557/PROC-0895-G03-06
, Energy payback times of PV modules and systems , 2009
, Life-cycle analyses of very-large scale PV systems using six types of PV modules , 2010, https://doi.org/10.1016/j.cap.2009.11.028
, Life cycle assessment of a wind farm and related externalities , 2000, https://doi.org/10.1016/S0960-1481(99)00123-8
, Climate change external cost appraisal of electricity generation systems from a life cycle perspective: the case of Greece , 2012, https://doi.org/10.1016/j.jclepro.2012.03.030
, (2010) parabolic trough reference plant for cost modeling with the Solar Advisor Model (SAM); NREL Report No , 2010, https://doi.org/10.2172/983729
, (2017) Energy and land use - Global land outlook working paper, UNCCD/IRENA , 2017
, A robust strategy for sustainable energy , 2005, https://doi.org/10.1353/eca.2006.0007
, World geothermal power generation in the period 2001-2005 , 2001, https://doi.org/10.1016/j.geothermics.2005.09.005
, Influence of site specific parameters on environmental performance of wind energy converters , 2012, https://doi.org/10.1016/j.egypro.2012.03.039
, Comparative life cycle energy, emission, and economic analysis of 100 kW nameplate wind power generation , 2012, https://doi.org/10.1016/j.renene.2011.06.003
, Assessing the lifecycle greenhouse gas emissions from solar PV and wind energy: a critical meta-survey , 2014, https://doi.org/10.1016/j.enpol.2013.10.048
, The potential of concentrating solar power in South Africa , 2009, https://doi.org/10.1016/j.enpol.2009.07.017
, Relocation, repatriation, and translocation of amphibians and reptiles: are they conservation strategies that work? Herpetologica 2012;47:336-50 , 2012
, An assessment of the published results of animal relocations , 2000, https://doi.org/10.1016/S0006-3207(00)00048-3
, Recommendations of independent science advisors for the California Desert Renewable Energy Conservation Plan (DRECP) , 2010
, Renewable energy development on California BLM lands: can we leverage mitigation into recovery for the desert tortoise? The Wildlife Society, Annual Meeting , 2010
, Draft programmatic environmental impact statement for solar energy development in six southwestern states; 2010. , 2010
, 2002 , 2002
, Impact of solar thermal power plants on water resources and electricity costs in the Southwest , 2009, https://doi.org/10.1109/NAPS.2009.5483989
, 2006 , 2006, https://doi.org/10.1115/ISEC2006-99082
, Paper no , 2006
, Environmental impact and pollution-related challenges of renewable wind energy paradigm - A review , 2019, https://doi.org/10.1016/j.scitotenv.2019.05.274
, Texas wind farm uses NASA radar to prevent bird deaths, Treehugger, http://www.digitaljournal.com/article/272061, [Accessed 2 August 2020] , 2020
, Changing wind-power landscapes: regional assessment of visual impact on landuse and population in Northern Jutland, Denmark , 2005, https://doi.org/10.1016/j.apenergy.2005.04.004
, Shadow-flicker modeling at Wild Horse, WA , 2003
, Visual assessment of off-shore wind turbine: the influence of distance, contrast, movement and social variables , 2007, https://doi.org/10.1016/j.renene.2006.03.009
,